More than a decade on since then, investigating the fundamental principles of matter and energy is exactly what Colquhoun is endeavoring to do in KEK’s Lattice QCD research group, where he has been conducting research for the past two years.
In particular, Colquhoun is interested in some of the most fundamental and perhaps strangest of all particles that compose our universe: quarks. These elementary particles are the constituents of protons and neutrons that in turn make up the nucleus of all atoms and therefore all the matter around us.
So why are quarks strange? Several reasons.
Quarks have only fractional electric charges, not whole numbers like all other particles with an electric charge. And they are confined together in protons and neutrons so tightly they can’t be pulled out for examination. This is because their interaction force does not decrease with distance when efforts are made to separate them. Hence the name given to this force is the strong force, one of the four fundamental forces in nature, the other three being the weak (interaction) force, the electromagnetic force, and gravity.
There are six types or flavors of quarks all exhibiting differences in their properties, such as their charge or mass. These types always interact in groups of flavors, never alone. And in keeping with their unusual nature, physicists have given them curious names: up, down, charm, strange, top, bottom.
A proton is made up of two up quarks and one down quark; the neutron is composed of two down quarks and one up quark. Up quarks carry a 2/3 positive electric charge and the down quark a 1/3 negative electric charge. Add these together and you get a positive electric charge of 1 for the proton and 0 electric charge for the neutron—something we knew before quarks were first postulated in the 1960s.
To further complicate matters, there also exist six antiquarks, which are really the same as their corresponding quarks but have their charges reversed. Actually, this is not so strange, as other elementary particles also have their antiparticles; still, this adds to the complexity of quarks when it comes to studying their role in nature.
If all this weren’t enough, researchers have found that as well as the different flavors that exist, each quark also carries one of three special charges. These charges have been named after the colors red, blue and green, while their opposite colors anti-red, anti-blue and anti-green are used for the corresponding antiquarks and can be thought of as magenta, cyan and yellow.
Note that when you mix together the colors in either group, you get something that is colorless. This is important because only colorless combinations can be observed. Even more interesting, quarks can change from one color charge to another, something that physicists like Colquhoun are investigating.
To study quarks and other elementary particles, experimental physicists use colliders to smash particles into each other, or they build detectors to view particles from space hitting Earth, and then analyze the results.
Theorists, on the other hand, use mathematical-based methods, including the use of supercomputers to simulate how particles interact. In Colquhoun’s case he simulates the interaction of quarks based on a theory of their strong interaction force. The name of this theory is quantum chromodynamics or just QCD. The name is derived from the Greek word for color: chromo.
It might help to think of QCD as analogous to quantum electrodynamics (QED): the quantum theory of the electromagnetic force. QED is used to visualize the interaction between charged particles, two electrons, for instance, as an exchange of forces in the form of photons. But in this case there is only one charge—positive or negative—to consider.
To explain the behavior of quarks in QCD theory, three kinds of charges must be taken into account. In addition, there are the force-carrying particles called gluons, which are analogous to the photons in QED, and which keep the quarks bound together in protons and neutrons. By assigning colors to both quarks and gluons in this way, it becomes easier to therorize what is happening as they interact.
Colquhoun’s doctorial thesis dealt with the properties of the bottom quark, one of the heaviest quarks.
“This is something that KEK’s Lattice QCD research group is also studying,” he says. “What’s more, KEK has an accelerator, which makes KEK an attractive center of particle research for me.” So he was delighted when his application to continue his post-doctorial research there was accepted.
He says one reason for researchers’ interest in the bottom quark is that it offers the possibility of finding discrepancies between the Standard Model of particle physics and what is observed in experimentation.
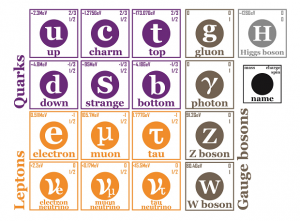
The Standard Model is our best understanding of particle physics. It includes all known particles and theorizes how they interact with each other.
The Standard Model theory can be thought of as physicists’ equivalent of chemists’ periodic table. It contains all known seventeen fundamental particles that make up matter, the forces that hold them together, and it describes how the particles interact with each other.
“Though the Standard Model is our best understanding of particle physics, it doesn’t explain or include everything known,” says Colquhoun. “Gravity and dark matter, for example, are missing. It’s also possible there may be other forces in nature beyond the strong and weak forces, gravity and electromagnetic force.”
In studying the bottom quark, he uses a simulation method called Lattice QCD. This utilizes a four-dimensional grid of equally spaced points connected by intersecting lines that represent the three dimensions of space plus the dimension of time.
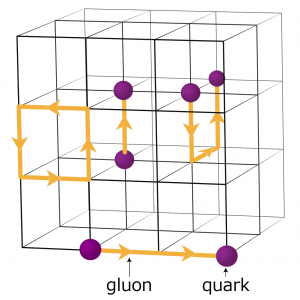
Diagram of a grid in lattice QCD, a simulation method used to calculate various particle interactions.
Mathematical values for quarks are placed on the intersections of the grid. In addition, more values are added for the lines connecting the quarks to represent the gluon forces that make interaction between quarks possible. Part of the work of lattice theorists involves writing different equations that enable this placement of values on the grid, depending on what is being investigated.
Through testing various configurations of grid setups for quarks and gluons, Colquhoun and his colleagues optimize their calculations by choosing configurations with the appropriate numbers of space and time points balanced against the amount of supercomputing time it takes to simulate the interactions between the particles.
“You want to get to a stage where you have as many points on the lattice as possible and still achieve a result in a year, say, rather than adding many more points which could then take five years to get a result,” he explains.
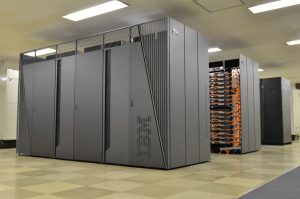
The Blue Gene/Q supercomputer used by the KEK Lattice QCD researchers to simulate particle interactions.
What the simulations are showing is that there are a lot of changes going on between the quarks and gluons inside a proton, for instance. “We have known for a long time that there are more than just valance or stable quarks,” he says. “There are also short-lived quark pairs called sea quarks continually coming into existence and decaying. So what we have is a dynamic situation which makes it a challenge to understand.”
Two specific areas of interest for Colquhoun is calculating more accurately the mass of the bottom quark and calculating how likely it is to change into an up quark.
However, the estimated mass of the bottom quark is too heavy to simulate in a straightforward way on a lattice. Instead, the masses of lighter quarks are simulated and then plotted out on a graph. This enables researchers to infer the behavior of a bottom quark and to extrapolate to its location on the graph, and so predict its properties together with its real mass.
When it comes to calculating a flavor change, Colquhoun and his colleagues employ a mathematical matrix called the Cabibbo-Kobayashi-Maskawa or CKM matrix that contains values on the probability that one quark flavor changes to another.
By simulating the behavior of probable changes and comparing the results with what has been measured in experiments, researchers are able to check and compare results of all the values in the CKM matrix precisely and in different ways. A deviation here from what is predicted would raise questions about current knowledge. And indeed some deviations in the values have been found when measuring the same quantities in different ways.
“The difference is not very large but something is going on,” notes Colquhoun. “It could be that we just need more data. Or we may have to reexamine the assumptions we have made when analyzing the experimental data. There again, perhaps there is something we have overlooked in our own theoretical calculations. Or, more exciting, these differences could indicate there is new physics to discover.”
The challenge for Colquhoun and his fellow researchers now is to find which of these answers is correct.